LGALS2 suppresses the progression of papillary thyroid carcinoma by regulating the PI3K/AKT pathway
Introduction
Thyroid carcinomas are the most common form of head and neck tumors and account for 95% of endocrine tumors (1). Of these, 70% of cases consist of the papillary thyroid cancer (PTC) subtype (2). While the treatment of PTC is generally associated with good prognostic outcomes, some patients ultimately develop metastases in their lymph nodes or distant sites that can contribute to recurrent tumor growth and associated mortality (3). Thyroid cancer rates have risen in recent years (4), highlighting a need to better define the molecular targets associated with this cancer type to aid in its diagnosis, prevention, and the prognostic evaluation of affected patients.
The LGALS (galectin) family consists of galactoside-binding glycoproteins harboring ~130 conserved amino acid carbohydrate recognition domains (CRDs) (5). To date, 15 LGALS have been identified in mammals (LGALS1-LGALS15), and they are expressed across a diverse range of both healthy and malignant cells. These proteins have also been linked to key pathological and physiological processes, including cellular adhesion, differentiation, intracellular signaling, inflammation, and tumor metastasis (6-8). Aberrant LGALS expression has been detected in many tumors and is associated with invasive and metastatic growth (9-11). Thijssen et al. (12) found LGALS expression was related to several cancer-associated indicators, including grade, clinical stage, and lymph node status. Accordingly, the prognostic relevance of LGALS proteins has been assessed in several cancers (13-16). However, the prognostic relevance of specific LGALS proteins in PTC remains to be established. The overexpression of LGALS2 was recently shown to be linked to better prognosis in human breast cancer (17). An in vivo CRISPR screens targeting disease-related immune genes identified LGALS2 as a candidate regulator in triple negative breast cancer (TNBC) involving immune escape, and blockade of LGALS2 using an inhibitory antibody successfully arrested tumor growth and reversed the immune suppression (18). A genome-wide CRISPR knockout screening approach identified LGALS2 were highly enriched in cells survived after sublethal H2O2 challenge, and LGALS2 overexpression decreased the proliferation of human colon tumor epithelial cells and blunted H2O2-induced STAT3 phosphorylation (19). The function and prognostic relevance of this LGALS family member, especially LGALS2 in PTC have yet to be established, and thus we designed the present study to gain insight into the role of LGALS2 in PTC.
We used an in vitro approach to examine the functional role of LGALS2, with appropriate constructs employed to knock down or overexpress this gene, followed by an assessment of the proliferative and apoptotic activity of LGALS2 cells. In addition, the influence of LGALS2 on in vivo tumor growth was assessed, and phosphatidylinositol-3-kinase/protein kinase B (PI3K/AKT) pathway activation was examined in LGALS2 overexpression or knockdown. Our results indicated that LGALS2 suppresses PTC tumor growth via PI3K/AKT pathway regulation. We present the following article in accordance with the ARRIVE reporting checklist (available at https://gs.amegroups.com/article/view/10.21037/gs-22-452/rc).
Methods
Tissue sample collection
Eighty pairs of tumor and paracancerous tissues from PTC patients were collected from July 2019 to March 2021. All patients had a confirmed pathological diagnosis of PTC and had not received radiotherapy or chemotherapy prior to the sample collection. All patients who participated in this study signed an informed consent form, and the study was approved by the Ethics Committee of The Second Affiliated Hospital of Nanchang University (No. 20210304076). This study was performed in accordance with the Declaration of Helsinki (as revised in 2013).
Cell culture and treatment
The human TPC-1 and BHT101 PTC cell lines were obtained from the American Type Culture Collection (VA, USA) and were cultured in Dulbecco’s modified Eagle’s medium (DMEM) containing 10% fetal bovine serum (FBS; Gibco, USA) and penicillin/streptomycin in a 5% CO2 incubator at 37 ℃. The media was exchanged every other day, and cells were passaged (1:3) every 6 days. LGALS2-specific and control siRNA constructs were obtained from Genepharma (Shanghai, China). An LGALS2 overexpression construct was produced by inserting the coding sequence for this gene into a pcDNA4.0 vector. Cells were grown until they reached 80–90% confluence and were then transfected with these constructs using Lipofectamine 2000 (Invitrogen, CA, USA) based on the manufacturer’s instructions. After 6 h, the media was exchanged for complete media. After 24–48 h, the cells were collected for further use.
Western immunoblotting
RIPA buffer (Beyotime, Beijing, China) supplemented with protease and phosphatase inhibitors (Genebase, Shanghai, China) was used to extract total protein from the cells, after which the proteins were quantified using a BCA Protein Assay kit (Thermo Scientific, USA). The protein samples (30 µg) were separated using 10–12% sodium dodecyl sulfate polyacrylamide gel electrophoresis (SDS-PAGE) and transferred onto polyvinylidene fluoride (PVDF) membranes (Millipore, MA, USA). Blots were probed with antibodies specific for LGALS2 (Abcam; #ab239501; 1:1,000), PI3K (Abcam; #ab32089; 1:10,000), AKT (Abcam; #ab8805; 1:10,000), p-AKT (Abcam; #ab38449; 1:10,000), or GAPDH (CST; #3686; 1:1,000) overnight at 4 ℃, followed by probing for 1 h with appropriate secondary antibodies at room temperature. Protein bands were then detected using an ECL Detection System (Amersham, NJ, USA). Samples were analyzed in triplicate, and GAPDH served as the normalization control. ImageJ (Rawak Software Inc., Stuttgart, Germany) was used to quantify the protein levels in individual samples.
Real-time quantitative polymerase chain reaction (qPCR)
Trizol (Invitrogen, USA) was used to extract the total cellular RNA, after which the cDNA was prepared with a BestarTM qPCR RT kit (DBI Bioscience, #2220, Germany). The primers were as follows: LGALS2-F 5'-CATGACGGGGGAACTTGAGG-3', LGALS2-R 5'-AGGTTCAGCTTGTCTGTCCC-3', GAPDH-F 5'-GACAGTCAGCCGCATCTTCT-3', and GAPDH-R 5'-GCGCCCAATACGACCAAATC-3'. BestarTM qPCR MasterMix (DBI Bioscience, #2043, Germany) was used to assess gene expression via qPCR, with GAPDH as the normalization control. Relative expression levels were detected via the 2−ΔΔCt method.
CCK-8 assay
The transfected cells were added to 96-well plates (2×103/well) and grown under normal conditions for 48 h, at the end of the time, 10 µL of CCK-8 solution (Dojindo Laboratories, Kumamoto, Japan) were added. The cells were then incubated for an additional 2 h. Absorbance at 450 nm was assessed using a Tecan microplate reader (Tecan Group, Ltd.) to detect cellular viability.
EdU uptake assays
Cellular proliferation was quantified with an EdU assay kit (Ribobio, Guangzhou, China). Briefly, cells were added to confocal plates (10×105/well) and incubated for 2 h with 50 µM EdU buffer at 37 ℃. After fixation for 30 min using 4% paraformaldehyde, cells were permeabilized for 20 min using 0.1% Triton X-100. Hoechst was used for nuclear counterstaining, and the cells were imaged via fluorescent microscopy.
Apoptosis cell death analyses
TPC-1 and BHT101 cells transfected with the appropriate vectors were added to 6-well plates and grown to 90% confluence, after which they were harvested and stained for 15 min using 10 µL of dye from an Annexin V-FluoresceinIsothiocyanate/Propidium Iodide (FITC/PI) apoptosis kit (Lianke Biotech Co. Ltd., Hangzhou, China) at room temperature in the dark. A flow cytometer (FACS Calibur, BD Biosciences, USA) was then used for the cell analysis.
Immunohistochemistry (IHC)
The samples were processed for IHC staining by deparaffinization, antigen retrieval, inactivation of endogenous peroxidase activity, blocking with goat serum (Gibco), and incubation overnight with primary anti-Ki67 (Abcam; #ab15580; 1:400) and an appropriate secondary antibody (Abcam; #ab205718; 1:4,000) in sequence. Sections were then imaged using a phase contrast microscope (Leica, Cat. #DMI 1).
Murine xenograft analyses
Nude athymic BALB/c mice (male, 4 weeks old) from the SLAC Laboratory Animal Co. (Shanghai, China) were used for these studies, which received approval from the Ethics Committee of of The Second Affiliated Hospital of Nanchang University (No. 20210304076) in accordance with the Guide for the Care and Use of Laboratory Animals (8th edition, NIH). A protocol was prepared before the study without registration. Animals were housed in a specific pathogen-free setting and were randomized into four groups (n=5/group). Xenograft tumors were generated by subcutaneous injection in the left flank of the mice with TPC-1 cells that had been stably transfected with pcDNA4.0 vector, pcDNA4.0-LGALS2 vector, CTRL‑siRNA, or LGALS2‑siRNA constructs (3×107 cells/mouse). The tumor volume and weight was monitored every third day for four weeks with calipers, with the volume being determined as follows: V = length × width2 × 0.5. The animals were euthanized after 28 days. The tumors were harvested, fixed for 24 h with 4% paraformaldehyde, and subsequently processed for IHC staining for Ki67 detection.
Statistical analysis
All experiments were performed at least three times. GraphPad Prism 7.0 (GraphPad, CA, USA) was used to analyze all data, which are displayed as means ± standard deviation (SD). The data were compared using t-tests and one-way ANOVAs. The associations between LGALS2 expression and clinicopathological features have been analyzed using Chi square test. Independent prognostic factors were established using a Cox proportional-hazards model. P<0.05 was the significance threshold.
Results
LGALS2 was downregulated in human PTC tissues and associated with patient outcomes
To explore the relevance of LGALS2 as a diagnostic biomarker in PTC, 80 paired tumor and paracancerous tissue samples were harvested from PTC patients. LGALS2 mRNA levels, as detected by qPCR, were significantly reduced in tumor tissues relative to paired normal tissues (P<0.0001; Figure 1A). Western immunoblotting confirmed similar results at the protein level (Figure 1B,1C). ROC curves revealed that LGALS2 offered value as a highly sensitive biomarker of PTC (AUC =0.9456, Figure 1D), suggesting the potential diagnostic utility of the loss of LGALS2 expression. Correlations between LGALS2 levels and PTC patient clinicopathological characteristics were also assessed, and higher LGALS2 mRNA levels were associated with a low TNM stage (Figure 2, Table 1). These results suggested that LGALS2 downregulation could be a prognostic biomarker in patients with PTC.
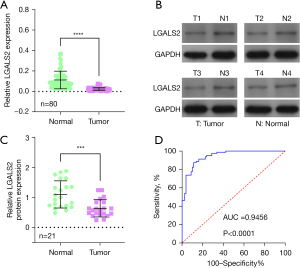
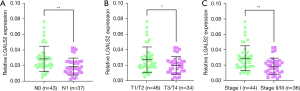
Table 1
Parameters | Group | N | Expression of LGALS2 | P value | |
---|---|---|---|---|---|
Low | High | ||||
Age (years) | ≤45 | 42 | 24 | 18 | 0.6855 |
>45 | 38 | 20 | 18 | ||
Gender | Female | 57 | 27 | 30 | 0.7521 |
Male | 23 | 10 | 13 | ||
Tumor size (cm) | <1 | 44 | 24 | 20 | 0.3687 |
≥1 | 36 | 16 | 20 | ||
N stage | N0 | 43 | 13 | 30 | 0.0020 |
N1 | 37 | 24 | 13 | ||
T stage | T1/T2 | 46 | 17 | 29 | 0.0029 |
T3/T4 | 34 | 24 | 10 | ||
M stage | I | 44 | 17 | 27 | 0.0060 |
II-III | 36 | 25 | 11 |
LGALS2, galectin 2; PTC, papillary thyroid carcinoma.
LGALS2 knockdown caused more rapid PTC cell proliferation in vitro
To further explore how LGALS2 shapes the development and progression of PTC, we knocked down and overexpressed LGALS2 in the BHT101 and TPC-1 PTC cell lines. The successful knockdown and overexpression of LGALS2 in these cells were validated by qPCR and western immunoblotting (Figure 3A,3B). Subsequently, the proliferation of these transfected cells was examined with CCK-8 and EdU uptake assays. We found that LGALS2 overexpression significantly impaired PTC cell proliferation relative to OE-NC control cells (Figure 3C), whereas knocking down LGALS2 enhanced the ability of these cells to proliferate relative to shCTRL transfection. The EdU assays similarly confirmed that LGALS2 knockdown enhanced the proliferative activity of PTC cells (Figure 3D,3E). These data thus confirmed the role of LGALS2 as a tumor suppressor gene in PTC.
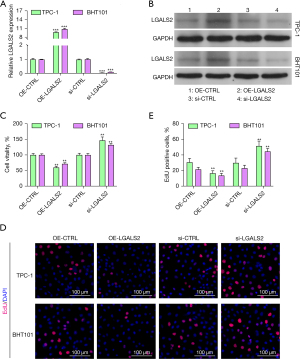
LGALS2 induced enhanced PTC cell apoptotic death through the PI3K/AKT pathway
Apoptotic cell death is an important regulator of PTC cell malignancy. We used a flow cytometry-based assay to explore the ability of LGALS2 to regulate apoptosis and thereby suppress tumorigenesis. The resultant data indicated that LGALS2 knockdown reduced the apoptotic death of PTC cells relative to control siRNA transfection, while LGALS2 overexpression resulted in higher apoptosis rates (Figure 4A,4B). LGALS2 is thus a driver of PTC cell apoptotic death in vitro. Given the hypothesis that LGALS2 can suppress PTC proliferative activity and survival through the suppression of AMPK/mTOR signaling, changes in p-PI3K and p-AKT levels were subsequently assessed by western immunoblotting. Reduced p-PI3K and p-AKT levels were detected following the overexpression of LGALS2, whereas higher levels of PI3K/AKT pathway activity were evident following sh-LGALS2 transfection (Figure 4C-4E). Thus, LGALS2 can suppress the progression of PTC through mechanisms at least partially related to PI3K/AKT pathway activation.
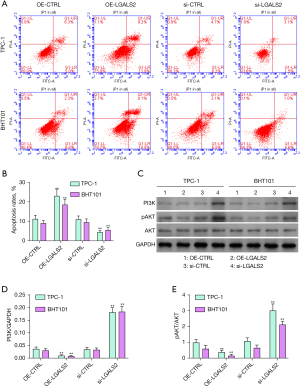
LGALS2 knockdown enhanced tumor growth via PI3K/AKT signaling activity
Lastly, a murine xenograft tumor model was established to explore the role of LGALS2 as a regulator of PTC tumor development in vivo. Nude athymic mice were subcutaneously implanted with TPC-1 cells stably transfected with LGALS2 overexpression or knockdown vectors or corresponding control constructs. Our results indicated that LGALS2 knockdown was associated with an increase in tumor cells relative to shCTRL transfection, whereas LGALS2 overexpression reduced tumor cells relative to the OE-CTRL group (Figure 5A,5B). IHC staining of the xenograft tumors from these mice revealed that LGALS2 knockdown significantly reduced the expression of the cancer differentiation-related marker Ki67 (Figure 5C,5D). Additionally, TUNEL staining revealed increased apoptotic tumor cell death in the OE-LGALS2 group relative to the OE-CTRL group, whereas LGALS2 knockdown suppressed such apoptotic activity (Figure 5E). Together, these results revealed that knockdown LGALS2 was sufficient to impair in vivo PTC cell growth and differentiation. Western immunoblotting was also used to examine p-PI3K and p-AKT levels in these tumors (Figure 5F), and the results were consistent with the in vitro data. As such, these findings indicate that LGALS2 can suppress PTC progression via PI3K/AKT pathway activation.
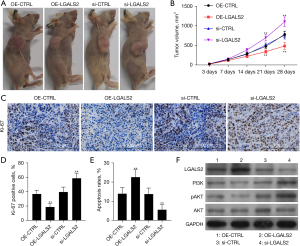
Discussion
LGALS family proteins are S-type lectins that bind to galactosidase-containing glycoproteins and harbor a conserved CRD sequence. They have emerged as important regulators of cellular processes such as adhesion, motility, survival, migration, and inflammation (20). Previous research efforts have revealed dysregulation of specific LGALS proteins in ovarian, prostate, liver, breast, bladder, and thyroid cancers (21-25).
Mechanistically, LGALS proteins can influence immunosuppressive pathway activity through interactions with specific receptors, influencing certain co-stimulatory pathways or controlling immune cell activation, differentiation, and survival (26,27). Abnormal LGALS expression has often been found to be associated with negative prognostic findings, including tumor grade, clinical stage, and lymph node status (28,29). LGALS1 and LGALS3, the best-studied members of this protein family, have repeatedly been shown to be associated with cancer patient outcomes. For example, higher levels of LGALS1 expression are associated with poorer prognosis in ovarian carcinoma (30), gingival squamous cell carcinoma (15), nasopharyngeal carcinoma (31), and pancreatic ductal adenocarcinoma (32). In contrast, data concerning the prognostic relevance of LGALS3 have been inconsistent and vary across tumor types. Ma et al. reported that anti-Gal-3 nanoparticles were effective mediators of drug delivery capable of increasing intracellular doxorubicin concentrations in vivo within thyroid cancer cells (33).
LGALS2 expression has previously been reported to be associated with gestational diabetes, a pregnancy-specific metabolic disorder (34). In an oncogenic setting, Jung et al. (35) determined that a lower level of LGALS2 expression was related to more advanced clinical stage and lymph node metastatic progression in patients diagnosed with gastric carcinoma, indicating that the loss of LGALS2 expression may contribute to more aggressive gastric tumor growth. However, few other studies have examined LGALS2 expression in human cancers. In grade III breast cancer, LGALS2 levels have been correlated with better overall survival (OS) (18), with similar prognostic relevance in breast cancer patients with basal-like, luminal B, TP53-mutated, HER2-overexpressing, and wild-type disease (36,37). Chetry et al. (17) further confirmed the value of LGALS2 as a predictor of more favorable breast cancer outcomes, emphasizing its value as a prognostic biomarker. However, no prior reports have examined the predictive relevance of LGALS2 expression in patients with PTC.
A detailed study of the molecular drivers of PTC pathogenesis have enabled the design of more reliable treatments for this cancer type (38). The PI3K/AKT pathway is among the most important known intracellular signaling mediators of essential processes, including proliferation, metabolic activity, protein synthesis, growth, and apoptotic death (39). Sustained PI3K/AKT pathway activity mediated by abnormal receptor tyrosine kinase activity can contribute to improved cellular proliferation and higher levels of vascularization (40). The activation of this pathway is also critical to PTC development and progression (41) with its deregulation contributing to altered sensitivity and resistance to particular therapeutic drugs (42). Further studies exploring the link between LGALS2 and the PI3K/AKT pathway may have important implications for PTC patient treatment and management, given that these data highlight a close relationship between the activity of this pathway and the expression of LGALS2, thereby maintaining PTC cell proliferation and survival.
Conclusions
Together, these data indicate that LGALS2 regulates PI3K/AKT pathway signaling to suppress PTC progression, suggesting that LGALS2 may be a valuable therapeutic and prognostic target in PTC treatment.
Acknowledgments
Funding: The research was supported by grants from the National Natural Science Foundation of China (No. 82160539).
Footnote
Reporting Checklist: The authors have completed the ARRIVE reporting checklist. Available at https://gs.amegroups.com/article/view/10.21037/gs-22-452/rc
Data Sharing Statement: Available at https://gs.amegroups.com/article/view/10.21037/gs-22-452/dss
Conflicts of Interest: All authors have completed the ICMJE uniform disclosure form (available at https://gs.amegroups.com/article/view/10.21037/gs-22-452/coif). The authors have no conflicts of interest to declare.
Ethical Statement: The authors are accountable for all aspects of the work, including ensuring that any questions related to the accuracy or integrity of any part of the work have been appropriately investigated and resolved. All patients who participated in this study signed an informed consent form, and the study was approved by the Ethics Committee of The Second Affiliated Hospital of Nanchang University (No. 20210304076). This study was performed in accordance with the Declaration of Helsinki (as revised in 2013). The animal experiment was approved by the Ethics Committee of The Second Affiliated Hospital of Nanchang University (No. 20210304076) in accordance with the Guide for the Care and Use of Laboratory Animals (8th edition, NIH).
Open Access Statement: This is an Open Access article distributed in accordance with the Creative Commons Attribution-NonCommercial-NoDerivs 4.0 International License (CC BY-NC-ND 4.0), which permits the non-commercial replication and distribution of the article with the strict proviso that no changes or edits are made and the original work is properly cited (including links to both the formal publication through the relevant DOI and the license). See: https://creativecommons.org/licenses/by-nc-nd/4.0/.
References
- Siegel RL, Miller KD, Fuchs HE, et al. Cancer statistics, 2022. CA Cancer J Clin 2022;72:7-33. [Crossref] [PubMed]
- Filetti S, Durante C, Hartl D, et al. Thyroid cancer: ESMO Clinical Practice Guidelines for diagnosis, treatment and follow-updagger. Ann Oncol 2019;30:1856-83. [Crossref] [PubMed]
- Coca-Pelaz A, Shah JP, Hernandez-Prera JC, et al. Papillary Thyroid Cancer-Aggressive Variants and Impact on Management: A Narrative Review. Adv Ther 2020;37:3112-28. [Crossref] [PubMed]
- Roman BR, Morris LG, Davies L. The thyroid cancer epidemic, 2017 perspective. Curr Opin Endocrinol Diabetes Obes 2017;24:332-6. [Crossref] [PubMed]
- Cooper DN. Galectinomics: finding themes in complexity. Biochim Biophys Acta 2002;1572:209-31. [Crossref] [PubMed]
- Wan L, Hsu YA, Wei CC, et al. Galectins in allergic inflammatory diseases. Mol Aspects Med 2021;79:100925. [Crossref] [PubMed]
- Garcia Caballero G, Kaltner H, Kutzner TJ, et al. How galectins have become multifunctional proteins. Histol Histopathol 2020;35:509-39. [PubMed]
- Johannes L, Jacob R, Leffler H. Galectins at a glance. J Cell Sci 2018;131:jcs208884. [Crossref] [PubMed]
- Chou FC, Chen HY, Kuo CC, et al. Role of Galectins in Tumors and in Clinical Immunotherapy. Int J Mol Sci 2018;19:430. [Crossref] [PubMed]
- Wang L, Zhao Y, Wang Y, et al. The Role of Galectins in Cervical Cancer Biology and Progression. Biomed Res Int 2018;2018:2175927. [Crossref] [PubMed]
- Fujihara S, Mori H, Kobara H, et al. Galectin-9 in cancer therapy. Recent Pat Endocr Metab Immune Drug Discov 2013;7:130-7. [Crossref] [PubMed]
- Thijssen VL, Heusschen R, Caers J, et al. Galectin expression in cancer diagnosis and prognosis: A systematic review. Biochim Biophys Acta 2015;1855:235-47. [PubMed]
- Chen L, Yao Y, Sun L, et al. Galectin-1 promotes tumor progression via NF-kappaB signaling pathway in epithelial ovarian cancer. J Cancer 2017;8:3733-41. [Crossref] [PubMed]
- Chou SY, Yen SL, Huang CC, et al. Galectin-1 is a poor prognostic factor in patients with glioblastoma multiforme after radiotherapy. BMC Cancer 2018;18:105. [Crossref] [PubMed]
- Noda Y, Kishino M, Sato S, et al. Galectin-1 expression is associated with tumour immunity and prognosis in gingival squamous cell carcinoma. J Clin Pathol 2017;70:126-33. [Crossref] [PubMed]
- Okada K, Shimura T, Suehiro T, et al. Reduced galectin-3 expression is an indicator of unfavorable prognosis in gastric cancer. Anticancer Res 2006;26:1369-76. [PubMed]
- Chetry M, Bhandari A, Feng R, et al. Overexpression of galectin2 (LGALS2) predicts a better prognosis in human breast cancer. Am J Transl Res 2022;14:2301-16. [PubMed]
- Ji P, Gong Y, Jin ML, et al. In vivo multidimensional CRISPR screens identify Lgals2 as an immunotherapy target in triple-negative breast cancer. Sci Adv 2022;8:eabl8247. [Crossref] [PubMed]
- Li H, Zhao L, Lau YS, et al. Genome-wide CRISPR screen identifies LGALS2 as an oxidative stress-responsive gene with an inhibitory function on colon tumor growth. Oncogene 2021;40:177-88. [Crossref] [PubMed]
- Li CH, Chang YC, Chan MH, et al. Galectins in Cancer and the Microenvironment: Functional Roles, Therapeutic Developments, and Perspectives. Biomedicines 2021;9:1159. [Crossref] [PubMed]
- Setayesh T, Colquhoun SD, Wan YY. Overexpression of Galectin-1 and Galectin-3 in hepatocellular carcinoma. Liver Res 2020;4:173-9. [Crossref] [PubMed]
- Yasinska IM, Sakhnevych SS, Pavlova L, et al. The Tim-3-Galectin-9 Pathway and Its Regulatory Mechanisms in Human Breast Cancer. Front Immunol 2019;10:1594. [Crossref] [PubMed]
- Kaur M, Kaur T, Kamboj SS, et al. Roles of Galectin-7 in Cancer. Asian Pac J Cancer Prev 2016;17:455-61. [Crossref] [PubMed]
- Li J, Vasilyeva E, Wiseman SM. Beyond immunohistochemistry and immunocytochemistry: a current perspective on galectin-3 and thyroid cancer. Expert Rev Anticancer Ther 2019;19:1017-27. [Crossref] [PubMed]
- Boutas I, Potiris A, Makrakis E, et al. The expression of Galectin-3 in breast cancer and its association with metastatic disease: a systematic review of the literature. Mol Biol Rep 2021;48:807-15. [Crossref] [PubMed]
- Eckardt V, Weber C, von Hundelshausen P. Glycans and Glycan-Binding Proteins in Atherosclerosis. Thromb Haemost 2019;119:1265-73. [Crossref] [PubMed]
- O'Sullivan JA, Carroll DJ, Bochner BS. Glycobiology of Eosinophilic Inflammation: Contributions of Siglecs, Glycans, and Other Glycan-Binding Proteins. Front Med (Lausanne) 2017;4:116. [Crossref] [PubMed]
- Dong R, Zhang M, Hu Q, et al. Galectin-3 as a novel biomarker for disease diagnosis and a target for therapy Int J Mol Med 2018;41:599-614. (Review). [PubMed]
- Pereira AR, Menezes Falcao L. Galectin-3, a prognostic marker--and a therapeutic target? Rev Port Cardiol 2015;34:201-8. [Crossref] [PubMed]
- Masoodi M, Shah ZA, Beigh AH, et al. Galectin-1 as a predictive biomarker in ovarian cancer. J Ovarian Res 2021;14:123. [Crossref] [PubMed]
- Chang SL, Li CF, Lin C, et al. Galectin-1 overexpression in nasopharyngeal carcinoma: effect on survival. Acta Otolaryngol 2014;134:536-42. [Crossref] [PubMed]
- Zhao X, Li H, Lyu S, et al. Single-cell transcriptomics reveals heterogeneous progression and EGFR activation in pancreatic adenosquamous carcinoma. Int J Biol Sci 2021;17:2590-605. [Crossref] [PubMed]
- Ma X, Li X, Shi J, et al. Host-Guest Polypyrrole Nanocomplex for Three-Stimuli-Responsive Drug Delivery and Imaging-Guided Chemo-Photothermal Synergetic Therapy of Refractory Thyroid Cancer. Adv Healthc Mater 2019;8:e1900661. [Crossref] [PubMed]
- Hepp P, Unverdorben L, Hutter S, et al. Placental Galectin-2 Expression in Gestational Diabetes: A Systematic, Histological Analysis. Int J Mol Sci 2020;21:2404. [Crossref] [PubMed]
- Jung JH, Kim HJ, Yeom J, et al. Lowered expression of galectin-2 is associated with lymph node metastasis in gastric cancer. J Gastroenterol 2012;47:37-48. [Crossref] [PubMed]
- Barrow H, Guo X, Wandall HH, et al. Serum galectin-2, -4, and -8 are greatly increased in colon and breast cancer patients and promote cancer cell adhesion to blood vascular endothelium. Clin Cancer Res 2011;17:7035-46. [Crossref] [PubMed]
- Miwa HE, Koba WR, Fine EJ, et al. Bisected, complex N-glycans and galectins in mouse mammary tumor progression and human breast cancer. Glycobiology 2013;23:1477-90. [Crossref] [PubMed]
- Xing M. Molecular pathogenesis and mechanisms of thyroid cancer. Nat Rev Cancer 2013;13:184-99. [Crossref] [PubMed]
- Hemmings BA, Restuccia DF. PI3K-PKB/Akt pathway. Cold Spring Harb Perspect Biol 2012;4:a011189. [Crossref] [PubMed]
- Alexandraki KI, Kaltsas G. Gastroenteropancreatic neuroendocrine tumors: new insights in the diagnosis and therapy. Endocrine 2012;41:40-52. [Crossref] [PubMed]
- Motti ML, Califano D, Troncone G, et al. Complex regulation of the cyclin-dependent kinase inhibitor p27kip1 in thyroid cancer cells by the PI3K/AKT pathway: regulation of p27kip1 expression and localization. Am J Pathol 2005;166:737-49. [Crossref] [PubMed]
- Martini M, De Santis MC, Braccini L, et al. PI3K/AKT signaling pathway and cancer: an updated review. Ann Med 2014;46:372-83. [Crossref] [PubMed]