Adipose regeneration and implications for breast reconstruction: update and the future
Background: breast cancer and the need for reconstruction
As the second most common cancer worldwide (11.9%), each year 1.7 million women receive a new diagnosis of breast cancer (1,2). While advances in treatment have meant an overall reduction in mortality [522,000 deaths per year (1)], the global burden of this disease continues to be significant (3). In Britain, the age standardised incidence and mortality remains one of the highest in the world with around 14,000 women losing their lives every year to this disease (4). For those that survive, the morbidity is life changing, with physical, emotional and psychological aspects requiring multidisciplinary management (3,5-8). The treatment of breast cancer has evolved significantly; our understanding of the pathophysiology and molecular aetiology has advanced the way in which primary and adjuvant therapy is designed and delivered (9,10). Personalised treatment is playing an essential role in improving life expectancy, which has doubled in the last 50 years (9).
In the UK, of the approximately 46,000 women diagnosed annually with breast cancer, around 40% undergo mastectomy as their primary therapeutic procedure (11,12) and 30% of these have either immediate or delayed reconstruction. NICE guidance recommends that all women undergoing breast cancer surgery should be offered immediate reconstruction at their initial operation (12,13). The caveat being that where patients are primarily undergoing adjuvant treatment, this can be delayed to such time that reconstruction becomes an option. The guidance goes on to recommend that even if reconstruction is not available locally, patients should be provided with all the options in order to make an informed choice (13). It may be surprising then, that of all women eligible; only 21% undergo immediate reconstruction (11). Patients who opt for reconstruction (either immediate or delayed) have been shown to have higher levels of well-being (emotionally and sexually) when compared to those undergoing mastectomy alone (12). It is important, therefore, as reconstructive techniques advance, to not only offer patients a choice of reconstructive options, but involve them in the decision making surrounding their treatment (Figure 1).
The earliest documented cases of breast cancer is The Edwin Smith papyrus (3,000 BC) which details eight patients with tumours of the breast that were clearly distinct from the surrounding tissue (14). Seen for many centuries as an incurable disease, early surgical intervention was often difficult and postponed for fear of shortening the life of the patient (14); Galen [120-200 AD] was one of the first to promote clear margins, identifying the ‘crab-like’ projections of the invading tumour (2,14). Work done by French surgeons Pare [1510-1590] and Cabrol [1549-1610] greatly informed surgical techniques by recognising the involvement of axillary nodes in the spread of disease (15). The advancements in anatomical understanding and development of mastectomy instruments in the 1500-1700s were limited until the invention and popularisation of anaesthesia by Morton in 1846 and asepsis by Lister in 1867 (2,9,14,16). The radical mastectomy developed by Halstead in the 1880s became the gold standard in breast cancer surgery for the next seven decades (9,14,17). Despite 75% of his patients having axillary node disease at the time of operation, Halstead was able to demonstrate a 40% cure rate at 5 years (14). The fear of leaving disease behind with less aggressive approaches meant that it wasn’t until the 1970s that alternatives to radical surgery were considered. Trials in Europe and the US were beginning to demonstrate comparable outcomes to Halstead could be achieved with breast conserving surgery (BCS) and radiotherapy (18-20).
The shifting attitude meant that reconstruction, which had been previously thought of as a ‘luxury operation’ became a more integrated part of the surgical management of these patients (2,21). Czerny is credited as the first to demonstrate mound reconstruction in a patient with benign disease by re-purposing a lipoma from their flank (22). The work done by Tazini and Ombredanne in the early 1900s demonstrated new techniques using local myocutaneous flaps to recreate the breast (23,24). This evolution continued throughout the 1900s; with the previously described tubed abdominal flap by Sir Harold Gilles, used in 1942 to reconstruct the breast following mastectomy (2). The modern era of breast reconstruction was heralded by the use of tissue expanders (25,26) and the development of the silicone implant in 1963 (2,9,14,23) making single stage reconstruction a reality.
Autologous reconstruction sought to provide a more aesthetic and natural alternative whilst avoiding the drawbacks of early breast implants such as capsular contracture, increased risk of infection and rupture and their incompatibility with radiotherapy (27-29). Following refinement of the abdominoplasty technique in the 1950s, the rectus abdominous myocutaneous flap became one of the workhorse flaps in breast reconstruction (30). The pioneering work by Hartrampf et al. in the early 1980s saw the refinement of the transverse rectus abdominous myocutaneous (TRAM) flap (23,31-34), which along with advances in microsurgical technique meant the development of tissue donor sites and advanced flap variations (35). As techniques progressed, so to did the focus; minimising donor site morbidity and functional loss. In the late 1980s and early 1990s the musculocutaneous perforator flap (DIEP) described by Kroll, Rosenfield, Koshima and Soeda offered the benefits of TRAM and latissimus dorsi flaps with significantly reduced donor-site morbidity (23,36-38). The refinement of free flaps continued and as understanding of vascular territories, imaging technology and surgical expertise advanced, surgeons were demonstrating successful autologous reconstruction with minimal donor site morbidity using a variety of free flaps including superficial inferior epigastric artery (SIEA) (39,40), inferior gluteal artery perforator (IGAP) (41-47) and transverse upper gracilis (TUG) (48-50). The shift away from radical mastectomy to BCS (51) reflects in part the advancement of detection and effectiveness of chemo-radiotherapy, but also the changing perspective and expectation of patients. Although free-flap reconstruction remains the gold standard; donor site morbidity, lengthy operative time and microsurgical expertise means it remains costly and drives research into tissue-engineered autologous solutions. In Melbourne for example, researchers are working to achieve an autologous reconstructive option that eliminates the need for donor site morbidity by using a biodegradable chamber with vascularised adipose tissue at its core (NEOPEC) (52).
There is increasing interest worldwide in the use of adipose tissue as an autologous filler for breast defects following oncologic resection (53,54). Fat transfer has the advantage of using the patient’s own tissue with minimal donor-site morbidity and eliminating the issues of foreign body reaction or rejection associated with synthetic implants. In recent years, fat transfer has been gaining interest in the fields of breast and plastic surgery, not only due to volume replacement, but also the beneficial effects on irradiated tissue (55). Despite expansion of fat transfer techniques, understanding of the underlying mechanisms remains lacking. The ASAPS/ASPS position statement in 2012 (56) gives a succinct overview of the issues surrounding contemporary fat transfer and stem cell therapies, and acknowledges the need for increased efforts into both basic science investigations and translation into evidence based clinical therapies. When replacing volume, fat graft survival is one of the major problems, with long term follow up studies revealing 20–70% volume loss (57). The lack of revascularisation of grafts commonly underpins resorption, which is particularly problematic in breast reconstruction, where larger volumes of fat are required. The result is that multiple procedures are required, with increased direct and hidden financial and emotional costs. Several groups are looking into ways to overcome this problem (58). In this article we provide an overview of relevant adipose physiology, discuss aspects of cell biology and biomaterials relevant to adipose tissue regeneration, and highlight the potential risks and future potential avenues for research.
Adipose physiology
As a specialised thermogenic organ responsible for the regulation of metabolism and energy, adipose tissue forms an essential part of normal homeostasis (59). Originating from the mesoderm (60), the ratio of each adipose tissue type varies with age; brown adipose tissue (BAT), which is highly metabolically active, is found in greater quantities in infants and has an important role in maintaining core body temperature (59,61,62). Responsible for adaptive non-shivering thermogenesis, the expression of uncoupling protein 1 (UCP1) allows BAT to dissipate energy as heat in order to maintain temperature balance (60,63,64). The decline of BAT continues throughout infancy and adolescence; in adults it is virtually undetectable having been almost entirely replaced with white adipose tissue (WAT) (61). WAT, which develops in multiple anatomical sites, acts as a store for energy in the form of triglycerides (64), and as an endocrine organ releasing adipokines in response to physiological stimuli (65,66). White mature adipocytes, which are round or oval in shape, have a diameter of between 25–200 µm depending on location and, along with a few elongated mitochondria, contain a single lipid droplet (62,65). Occurring in two stages; the transition from an immature to a mature adipocyte starts initially by the determination and differentiation of a multipotent stem cell into an adipoblast and pre-adipocyte, followed by its terminal differentiation into a mature adipocyte (60). It is these mature white adipocytes that surgeons use to create autologous reconstructions either as lipoaspirate in a free-fat graft or as the volume component of a muscle free flap. Found within the stromal vascular fraction (SVF) are heterogeneous subpopulations of cells, growth factors and cytokines which contribute to the secretory function of adipose tissue (67-69). Enzymatic digestion of SVF liberates cells with multilineage potential; pericytes and supra-adventitial cells (CD34+ CD146+/−CD45−) otherwise classified as adipose derived stem cells (ADSCs) (70).
Cell sources for adipose regeneration
As aforementioned, tissue engineering and stem cell research have the potential to revolutionise the future of reconstructive surgery by replacing tissue obviating need for donor site morbidity (71-73). Mesenchymal stem cells (MSC) are non-hematopoietic cells with multi-lineage potential and have been isolated from an increasingly varied number of tissues over the past 20 years (74). Having previously been isolated from umbilical cord blood, embryos and bone marrow, cells came at a high price and were often difficult to harvest and manipulate, and had variable quality as the cells aged (75-87). Balancing ease of harvest with yield and efficacy has been a delicate, and often difficult trade off which has prompted the scientific community to investigate alternative sources. In 2006, induced pluripotent stem cells (iPSC) were heralded as one potential solution; terminally differentiated cells were successfully regressed back into a state of pluripotency and demonstrated multilineage potential (88). Initially promising an abundance of easily accessible cells, the process of reprogramming the cells resulted in observed DNA errors, raising the question about the process and its effect on epigenetics (89,90). Focusing on the end tissue type, in 2002, researchers identified distinct stem cell populations within adult adipose tissue (91). ADSCs are multipotent MSC which exhibit characteristics similar to stem cells isolated from bone marrow stem cells (BMSC) (86,92-94). Compared to BMSCs however they are more readily accessible, easier to isolate and carry a significantly lower morbidity. Obtained from either excised fat or lipoaspirate (under local or general anaesthetic), adipose tissue yields between 100–1,000 times more stem cells per cubic centimetre than their bone marrow counterparts (95). The apparent abundance of these stem cell populations means relatively small reservoirs may have potentially significant yields of cells and therefore offer a reliable and easily accessible source of cells for tissue engineering (91,96,97). Moreover, ADSCs have a longer lifespan in culture than BMSCs prior to becoming senescent (94,98) which gives greater flexibility in the lab environment. ADSCs are naturally inclined to replenish lost volume by proliferation and maturation into adipocytes, which makes them ideally suited for adipose regeneration (99). However, identification of this stem cell population can pose a challenge; they are phenotypically very similar to MSCs of other origins and as such a combination of phenotype, morphology and secretory functions are required to distinguish this cell population as ADSCs (86,93,100,101). ADSCs have been shown to secrete several growth factors including vascular endothelial growth factor (VEG-f), hepatocyte growth factor, FGF-2, and insulin-like growth factor 1 (IGF-1) which play a key role in angiogenesis and adipose tissue regeneration. This highlights the potential importance of ADSCs in maintenance of transplanted tissue volume, an important consideration in adipose tissue engineering (102). Identifying the appropriate cell type for the creation of de novo breast tissue is only part of the challenge; the cells will ultimately need a scaffold in order to create structure and stability while they mature and provide support when they are first implanted.
Scaffolds for adipose regeneration
Current approaches to soft tissue regeneration include the use of fat grafts, natural or synthetic biomaterials to act as a filler material, and scaffolds to enable 2D and 3D cell culture and engineering of tissue. Biomaterials act as the biochemical and biophysical environment to tune the cell response for the specific tissue engineering requirements (103). Whether derived from human, animal or naturally occurring sources, biomaterials by design have a tendency towards low immunogenicity and degradation or incorporation into recipient tissue (104). Focusing on ADSCs as a potential cell source for adipose tissue engineering; a number of studies have examined their interaction with natural and synthetic scaffolds, but there remains a paucity of literature on clinical application (103,105). The aim of the ideal scaffold is universal regardless of tissue type; to produce ‘native like tissue’ with equivalent physiological and biochemical structure (biomimetic). Minimising structural and functional deviation is key, so scaffolds must balance the structural integrity to withstand physiological forces while remaining flexible enough to allow ingrowth of new tissue and constructive remodelling (106). Immunological characteristics of scaffolds must also be considered, to prevent pro-inflammatory responses where possible (106,107). Natural biomaterials show good biocompatibility, degradability and the ability to support tissue regeneration; and while synthetic materials are theorised to be more immunogenic, they have been shown to maintain mechanical integrity and assimilate over time (103,105).
Stem cell differentiation signalled by the extracellular microenvironment is a complex interplay of interactions, which is challenging to replicate (108). The material properties of potential scaffolds can influence lineage and differentiation as a result of their mechanical properties, mineralisation and chemical functionality (109). With the potential to function as extracellular matrix (ECM) in addition to supporting native ECM, biomaterials affect all facets of cell-scaffold interaction such as cell adhesion, proliferation and differentiation (110). The use of specific bioactive agents and material based delivery systems has developed the way in which scaffolds are viewed and our understanding regarding the control of stem cell differentiation and ultimate phenotype (111,112).
Scaffold composition
Natural & biological scaffolds
Natural materials explored to support adipogenesis include collagen, gelatine, silk and alginate (103,105,113-120). The application of silk, which is essentially a protein scaffold, has been extensively investigated across a number of engineered tissues. Silk fibrin demonstrates low immunogenicity and slow, controlled degradation, while maintaining adequate mechanical properties to allow cell seeding and new tissue formation, and has been shown to support adipogenesis in vitro and in vivo (103,105). Similar results were achieved in a comparable study, which examined the potential of lyophilised silk sponges and found they supported the adhesion of MSC in culture (121). Furthermore, the scaffolds allowed proliferation and infiltration of stem cells and supported remodelling when implanted in vivo (121). In keeping with providing patients with minimally invasive and low morbidity procedures, injectable scaffolds are gathering a greater research interest. Several biomaterial systems have been investigated to meet this clinical need; alginate/collagen microspheres, seeded with ASDCs are a promising injectable scaffold promoting the formation of fatty lobules after only 4 weeks in culture (122). A comparison of natural and synthetic hydrogels for use as an injectable scaffolds has been explored; alginate/o-carboxymethyl chitosan (O-CMC) and alginate/poly vinyl alcohol (PVA) with the inclusion of fibrin nanoparticles were compared (123). ADSCs demonstrated good adhesion, viability, proliferation and differentiation into adipocytes on these scaffolds. Cell differentiation studies of fibrin incorporated hydrogel scaffolds showed improved differentiation when compared to scaffolds without fibrin, which was confirmed by Oil Red O staining (123) (Table 1).
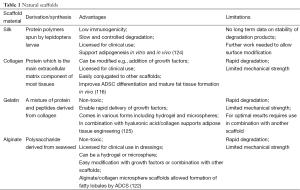
Full table
Biological scaffolds comprised of decellularised ECM are now widely used in preclinical and clinical tissue engineering studies (106). The preservation of structurally organised entities has been shown to act as a natural template and accommodate tissue regeneration (106); subcutaneous implantation of the adipose ECM in rats provoked a minimal inflammatory response and guided tissue remodelling and regeneration (126). Research evaluating the creation of more complex structures demonstrated that decellularised strategy for adipose tissue would provide a three-dimensional (3D) scaffold with adequate extracellular architecture (127). Seeding ADSCs on decellularised adipose tissue (DAT) demonstrated adipogenic differentiation, supporting the expression of the master regulators peroxisome-proliferator-activated receptor gamma (PPAR-γ2) and CCAAT/enhancer binding protein-alpha (CEBP-α), without the need for exogenous differentiation factors (127) (Table 2).
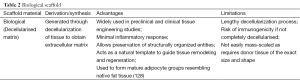
Full table
Synthetic scaffolds
Several synthetic polymers have been utilised for soft tissue regeneration including polygcolic acid (PLGA), polyethylene glycol (PEG), polycaprolactone (PCL) and poly-l-lactic acid (PLA) (129-132). Fibrous scaffolds are a particularly promising type of scaffold due to their ability to mimic the native ECM environment and guide de novo tissue formation (133). Freshly isolated ADSCs demonstrated adipogenic differentiation on polypropylene fibrous scaffolds within 19 days, with the expression of adipogenic marker PPARγ2 (133). Similarly electrospun nanofibrous scaffolds made of PLA maintained adipogenic differentiation of human BMSCs (133). PLGA fibres seeded with human MSC (hMSCs) encapsulated within alginate/chitosan hydrogel capsules showed adipogenic differentiation and maintenance of the adipogenic phenotype for 56 days in immunodeficient mice (129). When evaluating efficacy however, few studies have directly compared natural and synthetic materials for soft tissue regeneration. Examining the commercially available scaffolds currently on the market; type I collagen sponge, PLGA and hyaluronic acid gels were compared for their suitability for adipose tissue engineering (108). Each were harvested and examined histologically and immunohistochemically after 4 and 8 weeks of implantation in athymic mice (108) (Table 3).
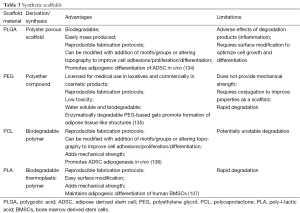
Full table
Current scaffolds
Of the readily available scaffolds; collagen sponges were found to be more suitable, having a higher expression of PPAR-γ2 and type I collagen than other two scaffolds. When combined with gelatin (collagen/gelatin mix), sponges modified with ADSCs and impregnated with basic fibroblast growth factor (bFGF) successfully demonstrated newly formed adipose tissue (125). Beyond promotion of adipogenesis, effects at the cellular level need to be taken into consideration; comparing natural fibrin glue with synthetic PCL as a framework demonstrates these differences in both cell yield and cell expression (136). After 6 weeks in vivo post seeding with ADSCs, adipose tissue and expression of adipogenic genes on the PCL scaffolds was significantly greater when compared to the fibrin scaffolds (136). These smaller studies demonstrate there is a broad variation in scaffold composition and structure, which can have a significant impact on tissue growth and quality. Production of large volume engineered adipose tissue extends beyond the scaffold material; it is essential that the graft maintain its size, shape and volume over time post implantation. Vascularisation is a common barrier discussed in research papers and as the pro-angiogenic properties of ADSCs have been demonstrated, supporting new vessel formation will be an essential for any scaffold using this cell type (138,139) (Table 4).
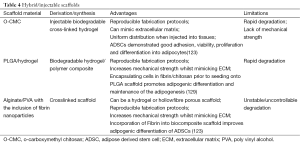
Full table
Scaffold free
The need for a scaffold has not been universally acknowledged however; research strategies examining scaffold free cell delivery is essentially an area that utilises one of three main techniques; use of single cells, cell sheet and micro tissues. Cell micro tissues have the advantage that they promote cell-to-cell and cell-to-matrix interactions (140) which is important in the formation of new tissue structure. Studies have demonstrated the ability of ADSCs to aggregate in cell culture and subsequently differentiate toward multiple cell lineage (140). It has been demonstrated that human ADSCs isolated from adipose tissue could be expanded and used to produce a 3D scaffold free micro tissue (141,142). The cells show uniformly positive expression for stem cell markers CD34, CD73, CD90, and CD105 are negative for CD19, CD14, and CD45, and were functionally inducible into adipocytes in appropriate medium (141). With the aim of enhancing adipogenesis by using a controlled delivery system; research has also explored the encapsulation of adipogenic factors within PLGA microspheres to deliver targeted growth within a transplanted fat graft (143,144). While this research is promising, translational barriers including scale-up limitations have led to an increasing interest in bioreactors and associated technologies to form controlled 3D assembly of stable adipose tissue (141).
Potential risks of and future challenges
Seen now as a reliable technique for autologous correction of volume loss and contour defects (145), stem cell populations within WAT have been shown to hold significant regenerative potential (73,146-148). This has resulted in the refinement of techniques which aim to increase the concentration of ADSCs in order to optimise graft retention and maximise therapeutic benefit (146,148). However it is the properties that make ADSCs so desirable which present the greatest concern because the cell characteristics useful for tissue regeneration and wound healing are shared with those features capable of promoting tumour growth and progression (148,149).
As the risk of dormant cells or remnant cancer post mastectomy or BCS cannot be entirely excluded, the oncological safety of tissue-engineered therapies must be appropriately considered. Recently there have been a number of clinical trials examining the safety and efficacy of ADSCs and related therapies in an attempt to evaluate their clinical potential (150,151). Cancer proliferation and progression relies on several factors to regulate and structure the tumour microenvironment (152). ADSCs demonstrate the production of factors that promote vascularisation, tissue growth, immune-modulation and cell recruitment (149). The expression of IL4, IL10, matrix metallopeptidase and SDF-1, in addition to pro-inflammatory mediators, has been shown to produce a microenvironment conducive to breast cancer recurrence (153). Additionally, the chemo-attraction of endothelial cells by production of VEGF and adipokines creates a microenvironment that can facilitate increased vascularisation, implicated in increased local recurrence risk (154). Conversely, the production of transforming growth factor (TGF) beta 1 and beta 2 has been shown to negatively affect cell differentiation and proliferation of breast cancer (155,156) demonstrating that ADSCs are only one part of a complex microenvironment. Increased metastatic potential has been further demonstrated in a study examining the co-culture of triple negative breast cancer and ADSCs; organ metastases were observed in the murine model in the ADSC group compared with none in the control (157).
The risk of de novo breast cancer is even less clear; the inhibition of hydrogen peroxide-induced cell death may play a factor in cell resistance to apoptosis and their propensity to develop down a carcinogenic line, however this hasn’t yet been fully demonstrated (158). While in vivo and in vitro studies have previously demonstrated the proliferative potential of ADSCs in the breast cancer environment (159); a recent meta-analysis of 2,428 patients found no significant difference in recurrence rates in the patient group who underwent fat grafting when compared to controls (160). Within this analysis however there was a single paper, which focused on in situ recurrence alone and found that the risk was 6 times greater in the group receiving fat grafts (161). Concerns regarding detection following breast surgery and fat grafting have been around since the late 1980s, and a number of studies have shown that there is no significant effect on radiographic surveillance following use of fat grafting (162). A retrospective study performed in the US found that all surgical procedures of the breast carried an increased risk of mammographic changes (163) and radiographic changes should be viewed in the context of patient specific factors.
Beyond the potential carcinogenesis risk, the complications of breast reconstruction must be taken into account. Issues with graft survival, volume loss and fat necrosis need to be considered. A systematic review found that 15.6% of patients reported a complication unrelated to recurrence demonstrating that non-malignant adverse events were not to be underestimated (164). Given the importance of reconstruction and follow-up in this patient group, it is essential that further studies examining both, the safety and efficacy of tissue engineered solutions, as well as effects on long term radiological monitoring, are carried out. It is only with further thorough, systematic studies, at a cellular and clinical level, that the true role of ADSCs in adipose tissue regeneration and cancer biology will be understood.
Summary
Surgeons and scientists face continued challenges in the coming years, to not only develop comparable and sustainable tissue engineered solutions with minimal donor site morbidity, but to prove their safety and efficacy. As the challenge to protect and improve patient quality of life continues to drive innovation forward, we cannot forget that these patients carry an inherent risk of potentially unseen or dormant breast cancer cells. The regenerative potential of ADSCs to generate de novo adipose tissue to replace lost breast volume has been well documented and is too good an opportunity to ignore. However, the use of stem cell therapies to expand and grow tissue for reconstruction must occur in the context of risk management. At each stage of clinical evaluation, patients must be fully informed of the benefits and potential risks. The autocrine and paracrine effects ADSCs must be fully investigated in rigorous clinical trials to evaluate their safety; and for those patients who have already undergone fat grafting following BCS, long-term follow up and careful monitoring is essential to examine the clinical impact of these therapies.
Acknowledgements
The authors would to acknowledge the ongoing support of the R&D department at ABMU and Swansea University.
Footnote
Conflicts of Interest: The authors have no conflicts of interest to declare.
References
- Ferlay J, Soerjomataram I, Dikshit R, et al. Cancer incidence and mortality worldwide: sources, methods and major patterns in GLOBOCAN 2012. Int J Cancer 2015;136:E359-86. [PubMed]
- Uroskie TW, Colen LB. A New Decade in Breast Reconstruction: History of Breast Reconstruction. Semin Plast Surg 2004;18:65-9. [PubMed]
- Li C, editor. Breast Cancer Epidemiology. New York, NY: Springer; 2010.
- McPherson K, Steel CM, Dixon JM. ABC of breast diseases. Breast cancer-epidemiology, risk factors, and genetics. BMJ 2000;321:624-8. [PubMed]
- Feiten S, Dünnebacke J, Heymanns J, et al. Breast cancer morbidity: questionnaire survey of patients on the long term effects of disease and adjuvant therapy. Dtsch Arztebl Int 2014;111:537-44. [PubMed]
- Robiolle C, Quillet A, Dagregorio G, et al. Patient-reported outcome of their breast reconstruction after mastectomy. Ann Chir Plast Esthet 2015;60:201-7. [PubMed]
- Ilfeld BM, Madison SJ, Suresh PJ, et al. Persistent postmastectomy pain and pain-related physical and emotional functioning with and without a continuous paravertebral nerve block: a prospective 1-year follow-up assessment of a randomized, triple-masked, placebo-controlled study. Ann Surg Oncol 2015;22:2017-25. [PubMed]
- Komenaka IK, Winton LM, Bouton ME, et al. Women's Impression of the Expected Breast Appearance and its Association with Breast Cancer Operations. Ann Surg Oncol 2015;22:2010-6. [PubMed]
- Wyld L, Audisio RA, Poston GJ. The evolution of cancer surgery and future perspectives. Nat Rev Clin Oncol 2015;12:115-24. [PubMed]
- Piper M, Peled AW, Sbitany H. Oncoplastic breast surgery: current strategies. Gland Surg 2015;4:154-63. [PubMed]
- Jeevan R, Cromwell D, Browne J, et al. National Mastectomy and Breast Reconstruction Audit 2011. The NHS information Centre 2011. Available online: http://www.hscic.gov.uk/catalogue/PUB02731/clin-audi-supp-prog-mast-brea-reco-2011-rep1.pdf
- Jeevan R, Cromwell DA, Browne JP, et al. Findings of a national comparative audit of mastectomy and breast reconstruction surgery in England. J Plast Reconstr Aesthet Surg 2014;67:1333-44. [PubMed]
- Harnett A, Smallwood J, Titshall V, et al. Diagnosis and treatment of early breast cancer, including locally advanced disease--summary of NICE guidance. BMJ 2009.b438. [PubMed]
- Cotlar AM, Dubose JJ, Rose DM. History of surgery for breast cancer: radical to the sublime. Current Surgery 2003;60:329-37. [PubMed]
- Sakorafas GH. The origins of radical mastectomy. AORN J 2008;88:605-8. [PubMed]
- Ellis H. The first “antiseptic” operation. J Perioper Pract 2015;25:87-8. [PubMed]
- Chapman-Jackson ED, Griner D, Brzezienski MA. Circumvertical mastectomy incision: refinement in the surgical scar of implant-based breast reconstruction. Ann Plast Surg 2014;72:S97-102. [PubMed]
- Fisher B, Anderson S, Bryant J. Twenty-Year Follow-up of a Randomized Trial Comparing Total Mastectomy, Lumpectomy, and Lumpectomy plus Irradiation for the Treatment of Invasive Breast Cancer. N Engl J Med 2002;347:1233-41. [PubMed]
- Hermann RE, Esselstyn CB, Crile G, et al. Results of conservative operations for breast cancer. Arch Surg 1985;120:746-51. [PubMed]
- Crile G Jr. Treatment of breast cancer: past, present, and future. Compr Ther 1983;9:38-40. [PubMed]
- Chagpar AB. Advances in the management of localized breast cancer: an overview. J Ky Med Assoc 2004;102:202-8. [PubMed]
- Goldwyn RM. Vincenz Czerny and the beginnings of breast reconstruction. Plast Reconstr Surg 1978;61:673-81. [PubMed]
- Champaneria MC, Wong WW, Hill ME, et al. The evolution of breast reconstruction: a historical perspective. World J Surg 2012;36:730-42. [PubMed]
- Teimourian B, Adham MN. Louis Ombredanne and the origin of muscle flap use for immediate breast mound reconstruction. Plast Reconstr Surg 1983;72:905-10. [PubMed]
- Ward J, Cohen IK, Knaysi GA, et al. Immediate Breast Reconstruction with Tissue Expansion. Plast Reconstr Surg 1987;80:559-66. [PubMed]
- Radovan C. Breast reconstruction after mastectomy using the temporary expander. Plast Reconstr Surg 1982;69:195-208. [PubMed]
- Holmes JD. Capsular contracture after breast reconstruction with tissue expansion. Br J Plast Surg 1989;42:591-4. [PubMed]
- Virden CP, Dobke MK, Stein P, et al. Subclinical infection of the silicone breast implant surface as a possible cause of capsular contracture. Aesthetic Plast Surg 1992;16:173-9. [PubMed]
- Pajkos A, Deva AK, Vickery K. Detection of Subclinical Infection in Signi cant Breast Implant Capsules. Plast Reconstr Surg 2003;111:1605-11. [PubMed]
- Mathes SJ, Bostwick J. A rectus abdominis myocutaneous flap to reconstruct abdominal wall defects. Br J Plast Surg 1977;30:282-3. [PubMed]
- Grotting JC, Beckenstein MS, Arkoulakis NS. The art and science of autologous breast reconstruction. Breast J 2003;9:350-60. [PubMed]
- Hartrampf CR, Scheflan M, Black PW. Breast reconstruction with a transverse abdominal island flap. Plast Reconstr Surg 1982;69:216-25. [PubMed]
- Robbins TH. Rectus abdominis myocutaneous flap for breast reconstruction. Aust N Z J Surg 1979;49:527-30. [PubMed]
- Robbins TH. Post-mastectomy breast reconstruction using a rectus abdominis musculocutaneous island flap. Br J Plast Surg 1981;34:286-90. [PubMed]
- Tamai S. History of Microsurgery. Plast Reconstr Surg 2009;124:e282-94. [PubMed]
- Geddes CR, Morris SF, Neligan PC. Perforator Flaps: Evolution, Classification, and Applications. Ann Plast Surg 2003;50:90-9. [PubMed]
- Koshima I, Soeda S. Inferior epigastric artery skin flaps without rectus abdominis muscle. Br J Plast Surg 1989;42:645-8. [PubMed]
- Allen RJ, Treece P. Deep Inferior Epigastric Perforator Flap for Breast Reconstruction. Ann Plast Surg 1994;32:32-8. [PubMed]
- Coroneos CJ, Heller AM, Voineskos SH, et al. SIEA versus DIEP Arterial Complications: A Cohort Study. Plast Reconstr Surg 2015;135:802e-7e. [PubMed]
- Spiegel AJ, Khan FN. An intraoperative algorithm for use of the SIEA flap for breast reconstruction. Plast Reconstr Surg 2007;120:1450-9. [PubMed]
- LoTempio MM, Allen RJ. Breast reconstruction with SGAP and IGAP flaps. Plast Reconstr Surg 2010;126:393-401. [PubMed]
- Fujino T, Abe O, Enomoto K. Primary reconstruction of the breast by free myocutaneous gluteal flap. Int Adv Surg Oncol 1981;4:127-43. [PubMed]
- Fujino T, Harasina T, Aoyagi F. Reconstruction for aplasia of the breast and pectoral region by microvascular transfer of a free flap from the buttock. Plast Reconstr Surg 1975;56:178-81. [PubMed]
- Paletta CE, Bostwick J 3rd, Nahai F. The Inferior Gluteal Free Flap in Breast Reconstruction. Plast Reconstr Surg 1989;84:875-83; discussion 884-5. [PubMed]
- Allen RJ, Tucker C. Superior gluteal artery perforator free flap for breast reconstruction. Plast Reconstr Surg 1995;95:1207-12. [PubMed]
- Allen RJ, Levine JL, Granzow JW. The in-the-crease inferior gluteal artery perforator flap for breast reconstruction. Plast Reconstr Surg 2006;118:333-9. [PubMed]
- Guerra AB, Metzinger SE, Bidros RS, et al. Breast reconstruction with gluteal artery perforator (GAP) flaps: a critical analysis of 142 cases. Ann Plast Surg 2004;52:118-25. [PubMed]
- Schoeller T, Wechselberger G. Breast reconstruction by the free transverse gracilis (TUG) flap. Br J Plast Surg 2004;57:481-2. [PubMed]
- McCulley SJ, Macmillan RD, Rasheed T. Transverse Upper Gracilis (TUG) flap for volume replacement in breast conserving surgery for medial breast tumours in small to medium sized breasts. J Plast Reconstr Aesthet Surg 2011;64:1056-60. [PubMed]
- Peek A, Müller M, Exner K. The free gracilis perforator flap for autologous breast reconstruction. Handchir Mikrochir Plast Chir 2002;34:245-50. [PubMed]
- Biazus JV, Falcão CC, Parizotto AC, et al. Immediate Reconstruction with Autologous fat Transfer Following Breast-Conserving Surgery. Breast J 2015;21:268-75. [PubMed]
- Mountford P. Neopec - Promising Preclinical Breast Reconstruction Research in Clinical Study in Melbourne. Australasian Biotechnology. Australas Biotechnol 2010;20:46.
- Chan CW, McCulley SJ, Macmillan RD. Autologous fat transfer--a review of the literature with a focus on breast cancer surgery. J Plast Reconstr Aesthet Surg 2008;61:1438-48. [PubMed]
- Ross RJ, Shayan R, Mutimer KL, et al. Autologous fat grafting: current state of the art and critical review. Ann Plast Surg 2014;73:352-7. [PubMed]
- Shukla L, Morrison WA, Shayan R. Adipose-derived stem cells in radiotherapy injury: a new frontier. Front Surg 2015;2:1. [PubMed]
- Eaves FF 3rd, Haeck PC, Rohrich RJ. ASAPS/ASPS Position Statement on Stem Cells and Fat Grafting. Plast Reconstr Surg 2012;129:285-7. [PubMed]
- Eto H, Kato H, Suga H, et al. The fate of adipocytes after nonvascularized fat grafting: evidence of early death and replacement of adipocytes. Plast Reconstr Surg 2012;129:1081-92. [PubMed]
- Findlay MW, Dolderer JH, Trost N, et al. Tissue-engineered breast reconstruction: bridging the gap toward large-volume tissue engineering in humans. Plast Reconstr Surg 2011;128:1206-15. [PubMed]
- Lee YH, Jung YS, Choi D. Recent advance in brown adipose physiology and its therapeutic potential. Exp Mol Med 2014;46:e78. [PubMed]
- Algire C, Medrikova D, Herzig S. White and brown adipose stem cells: from signaling to clinical implications. Biochim Biophys Acta 2013;1831:896-904.
- Virtanen KA, Lidell ME, Orava J, et al. Functional Brown Adipose Tissue in Healthy Adults. N Engl J Med 2009;360:1518-25. [PubMed]
- Boon MR, van Marken Lichtenbelt WD. Brown Adipose Tissue: A Human Perspective. Handb Exp Pharmacol 2015;1-19. Handb Exp Pharmacol 2015. [Epub ahead of print].
- Oelkrug R, Polymeropoulos ET, Jastroch M. Brown adipose tissue: physiological function and evolutionary significance. J Comp Physiol B 2015;185:587-606. [PubMed]
- Tiraby C, Tavernier G, Lefort C, et al. Acquirement of brown fat cell features by human white adipocytes. J Biol Chem 2003;278:33370-6. [PubMed]
- Qian S, Huang H, Tang Q. Brown and beige fat: the metabolic function, induction, and therapeutic potential. Front Med 2015;9:162-72. [PubMed]
- Frayn KN, Karpe F, Fielding BA, et al. Integrative physiology of human adipose tissue. Int J Obes Relat Metab Disord 2003;27:875-88. [PubMed]
- Palumbo P, Miconi G, Cinque B, et al. In vitro evaluation of different methods of handling human liposuction aspirate and their effect on adipocytes and adipose derived stem cells. J Cell Physiol 2015;230:1974-81. [PubMed]
- Gimble JM, Bunnell BA, Chiu ES, et al. Concise review: Adipose-derived stromal vascular fraction cells and stem cells: let's not get lost in translation. Stem Cells 2011;29:749-54. [PubMed]
- Johal KS, Lees VC, Reid AJ. Adipose-derived stem cells: selecting for translational success. Regen Med 2015;10:79-96. [PubMed]
- Silva KR, Liechocki S, Carneiro JR, et al. Stromal-vascular fraction content and adipose stem cell behavior are altered in morbid obese and post bariatric surgery ex-obese women. Stem Cell Res Ther 2015;6:72. [PubMed]
- Fuchs E, Segre JA. Stem cells: a new lease on life. Cell 2000;100:143-55. [PubMed]
- Mimeault M, Hauke R, Batra SK. Stem cells: a revolution in therapeutics-recent advances in stem cell biology and their therapeutic applications in regenerative medicine and cancer therapies. Clin Pharmacol Ther 2007;82:252-64. [PubMed]
- Kim EH, Heo CY. Current applications of adipose-derived stem cells and their future perspectives. World J Stem Cells 2014;6:65-8. [PubMed]
- Dominici M, Le Blanc K, Mueller I, et al. Minimal criteria for defining multipotent mesenchymal stromal cells. The International Society for Cellular Therapy position statement. Cytotherapy 2006;8:315-7. [PubMed]
- Thomson JA, Itskovitz-Eldor J, Shapiro SS, et al. Embryonic stem cell lines derived from human blastocysts. Science 1998;282:1145-7. [PubMed]
- da Silva Meirelles L, Caplan AI, Nardi NB. In search of the in vivo identity of mesenchymal stem cells. Stem Cells 2008;26:2287-99. [PubMed]
- Asano T, Sasaki K, Kitano Y, et al. In vivo tumor formation from primate embryonic stem cells. Methods Mol Biol 2006;329:459-67. [PubMed]
- Kuethe F, Richartz BM, Kasper C, et al. Autologous intracoronary mononuclear bone marrow cell transplantation in chronic ischemic cardiomyopathy in humans. Int J Cardiol 2005;100:485-91. [PubMed]
- Chen J, Zhang ZG, Li Y, et al. Intravenous administration of human bone marrow stromal cells induces angiogenesis in the ischemic boundary zone after stroke in rats. Circ Res 2003;92:692-9. [PubMed]
- Jiang Y, Mishima H, Sakai S, et al. Gene expression analysis of major lineage-defining factors in human bone marrow cells: effect of aging, gender, and age-related disorders. J Orthop Res 2008;26:910-7. [PubMed]
- Rebelatto CK, Aguiar AM, Moretão MP, et al. Dissimilar differentiation of mesenchymal stem cells from bone marrow, umbilical cord blood, and adipose tissue. Exp Biol Med (Maywood) 2008;233:901-13. [PubMed]
- Zhou S, Greenberger JS, Epperly MW, et al. Age-related intrinsic changes in human bone-marrow-derived mesenchymal stem cells and their differentiation to osteoblasts. Aging Cell 2008;7:335-43. [PubMed]
- Fehrer C, Lepperdinger G. Mesenchymal stem cell aging. Exp Gerontol 2005;40:926-30. [PubMed]
- Ren J, Wang H, Tran K, et al. Human bone marrow stromal cell confluence: effects on cell characteristics and methods of assessment. Cytotherapy 2015;17:897-911. [PubMed]
- Zhu Y, Liu T, Song K, et al. Adipose-derived stem cell: a better stem cell than BMSC. Cell Biochem Funct 2008;26:664-75. [PubMed]
- Wagner W, Wein F, Seckinger A, et al. Comparative characteristics of mesenchymal stem cells from human bone marrow, adipose tissue, and umbilical cord blood. Exp Hematol 2005;33:1402-16. [PubMed]
- Locke M, Windsor J, Dunbar PR. Human adipose-derived stem cells: isolation, characterization and applications in surgery. ANZ J Surg 2009;79:235-44. [PubMed]
- Csobonyeiova M, Polak S, Koller J, et al. Induced pluripotent stem cells and their implication for regenerative medicine. Cell Tissue Bank 2015;16:171-80. [PubMed]
- Hayden EC. Stem cells: The growing pains of pluripotency. Nature 2011;473:272-4. [PubMed]
- Laurent LC, Ulitsky I, Slavin I, et al. Dynamic changes in the copy number of pluripotency and cell proliferation genes in human ESCs and iPSCs during reprogramming and time in culture. Cell Stem Cell 2011;8:106-18. [PubMed]
- Zuk PA, Zhu M, Ashjian P, et al. Human adipose tissue is a source of multipotent stem cells. Mol Biol Cell 2002;13:4279-95. [PubMed]
- De Ugarte DA, Morizono K, Elbarbary A, et al. Comparison of multi-lineage cells from human adipose tissue and bone marrow. Cells Tissues Organs 2003;174:101-9. [PubMed]
- Gronthos S, Franklin DM, Leddy HA, et al. Surface protein characterization of human adipose tissue-derived stromal cells. J Cell Physiol 2001;189:54-63. [PubMed]
- Kern S, Eichler H, Stoeve J, et al. Comparative analysis of mesenchymal stem cells from bone marrow, umbilical cord blood, or adipose tissue. Stem Cells 2006;24:1294-301. [PubMed]
- Fraser JK, Wulur I, Alfonso Z, et al. Fat tissue: an underappreciated source of stem cells for biotechnology. Trends Biotechnol 2006;24:150-4. [PubMed]
- Zuk PA, Zhu M, Mizuno H, et al. Multilineage cells from human adipose tissue: implications for cell-based therapies. Tissue Eng 2001;7:211-28. [PubMed]
- Jurgens WJ, Oedayrajsingh-Varma MJ, Helder MN, et al. Effect of tissue-harvesting site on yield of stem cells derived from adipose tissue: implications for cell-based therapies. Cell Tissue Res 2008;332:415-26. [PubMed]
- Yoshimura K, Shigeura T, Matsumoto D, et al. Characterization of freshly isolated and cultured cells derived from the fatty and fluid portions of liposuction aspirates. J Cell Physiol 2006;208:64-76. [PubMed]
- Hong L, Peptan IA, Colpan A, et al. Adipose tissue engineering by human adipose-derived stromal cells. Cells Tissues Organs 2006;183:133-40. [PubMed]
- Mitchell JB, McIntosh K, Zvonic S, et al. Immunophenotype of human adipose-derived cells: temporal changes in stromal-associated and stem cell-associated markers. Stem Cells 2006;24:376-85. [PubMed]
- Katz AJ, Tholpady A, Tholpady SS, et al. Cell surface and transcriptional characterization of human adipose-derived adherent stromal (hADAS) cells. Stem Cells 2005;23:412-23. [PubMed]
- Nan H, Huang J, Li H, et al. Assessment of biological characteristics of adipose tissue-derived stem cells co-labeled with Molday ION Rhodamine B™ and green fluorescent protein in vitro. Mol Med Rep 2013;8:1446-52. [PubMed]
- Bellas E, Lo TJ, Fournier EP, et al. Injectable silk foams for soft tissue regeneration. Adv Healthc Mater 2015;4:452-9. [PubMed]
- Banyard DA, Bourgeois JM, Widgerow AD, et al. Regenerative biomaterials: a review. Plast Reconstr Surg 2015;135:1740-8. [PubMed]
- Bellas E, Panilaitis BJ, Glettig DL, et al. Sustained volume retention in vivo with adipocyte and lipoaspirate seeded silk scaffolds. Biomaterials 2013;34:2960-8. [PubMed]
- Keane TJ, Swinehart IT, Badylak SF. Methods of tissue decellularization used for preparation of biologic scaffolds and in vivo relevance. Methods 2015;84:25-34. [PubMed]
- Bai S, Han H, Huang X, et al. Silk scaffolds with tunable mechanical capability for cell differentiation. Acta Biomater 2015;20:22-31. [PubMed]
- Itoi Y, Takatori M, Hyakusoku H, et al. Comparison of readily available scaffolds for adipose tissue engineering using adipose-derived stem cells. J Plast Reconstr Aesthet Surg 2010;63:858-64. [PubMed]
- Phadke A, Chang CW, Varghese S. Functional Biomaterials for Controlling Stem Cell Differentiation. In: Roy K, editor. Biomaterials as Stem Cell Niche. Berlin Heidelberg: Springer; 2010:19-44.
- Wei Y, Hu Y, Hao W, et al. A novel injectable scaffold for cartilage tissue engineering using adipose-derived adult stem cells. J Orthop Res 2008;26:27-33. [PubMed]
- Kumar VA, Taylor NL, Shi S, et al. Self-assembling multidomain peptides tailor biological responses through biphasic release. Biomaterials 2015;52:71-8. [PubMed]
- Li Y, Meng H, Liu Y, et al. Fibrin gel as an injectable biodegradable scaffold and cell carrier for tissue engineering. ScientificWorldJournal 2015;2015:685690.
- Zhu Y, Liu T, Song K, et al. Collagen–chitosan polymer as a scaffold for the proliferation of human adipose tissue-derived stem cells. J Mater Sci Mater Med 2009;20:799-808. [PubMed]
- Zhou Y, Yan Z, Zhang H, et al. Expansion and Delivery of Adipose-Derived Mesenchymal Stem Cells on Three Microcarriers for Soft Tissue Regeneration. Tissue Eng Part A 2011;17:2981-97. [PubMed]
- Foubert P, Barillas S, Gonzalez AD, et al. Uncultured adipose-derived regenerative cells (ADRCs) seeded in collagen scaffold improves dermal regeneration, enhancing early vascularization and structural organization following thermal burns. Burns 2015;41:1504-16. [PubMed]
- Ferraro GA, De Francesco F, Nicoletti G, et al. Human adipose CD34+ CD90+ stem cells and collagen scaffold constructs grafted in vivo fabricate loose connective and adipose tissues. J Cell Biochem 2013;114:1039-49. [PubMed]
- Natesan S, Baer DG, Walters TJ, et al. Adipose-derived stem cell delivery into collagen gels using chitosan microspheres. Tissue Eng Part A 2010;16:1369-84. [PubMed]
- Davidenko N, Campbell JJ, Thian ES, et al. Collagen–hyaluronic acid scaffolds for adipose tissue engineering. Acta Biomater 2010;6:3957-68. [PubMed]
- Choi JH, Bellas E, Vunjak-Novakovic G. Adipogenic Differentiation of Human Adipose-Derived Stem Cells on 3D Silk Scaffolds. Methods Mol Biol 2011;702:319-30. [PubMed]
- Choi JH, Gimble JM, Lee K, et al. Adipose Tissue Engineering for Soft Tissue Regeneration. Tissue Eng Part B Rev 2010;16:413-26. [PubMed]
- Rnjak-Kovacina J, Wray LS, Burke KA, et al. Lyophilized Silk Sponges: A Versatile Biomaterial Platform for Soft Tissue Engineering. ACS Biomater Sci Eng 2015;1:260-70. [PubMed]
- Yao R, Zhang R, Lin F, et al. Injectable cell/hydrogel microspheres induce the formation of fat lobule-like microtissues and vascularized adipose tissue regeneration. Biofabrication 2012;4:045003. [PubMed]
- Jaikumar D, Sajesh KM, Soumya S, et al. Injectable alginate-O-carboxymethyl chitosan/nano fibrin composite hydrogels for adipose tissue engineering. Int J Biol Macromol 2015;74:318-26. [PubMed]
- Mauney JR, Nguyen T, Gillen K, et al. Engineering adipose-like tissue in vitro and in vivo utilizing human bone marrow and adipose-derived mesenchymal stem cells with silk fibroin 3D scaffolds. Biomaterials 2007;28:5280-90. [PubMed]
- Ito R, Morimoto N, Liem PH, et al. Adipogenesis using human adipose tissue-derived stromal cells combined with a collagen/gelatin sponge sustaining release of basic fibroblast growth factor. J Tissue Eng Regen Med 2014;8:1000-8. [PubMed]
- Wu I, Nahas Z, Kimmerling KA, et al. An injectable adipose matrix for soft-tissue reconstruction. Plast Reconstr Surg 2012;129:1247-57. [PubMed]
- Flynn LE. The use of decellularized adipose tissue to provide an inductive microenvironment for the adipogenic differentiation of human adipose-derived stem cells. Biomaterials 2010;31:4715-24. [PubMed]
- Wang JQ, Fan J, Gao JH, et al. Comparison of in vivo adipogenic capabilities of two different extracellular matrix microparticle scaffolds. Plast Reconstr Surg 2013;131:174e-87e. [PubMed]
- Morgan SM, Ainsworth BJ, Kanczler JM, et al. Formation of a human-derived fat tissue layer in P(DL)LGA hollow fibre scaffolds for adipocyte tissue engineering. Biomaterials 2009;30:1910-7. [PubMed]
- Wang X, Sui S, Yan Y, et al. Design and Fabrication of PLGA Sandwiched Cell/Fibrin Constructs for Complex Organ Regeneration. J Bioact Compat Polym 2010;25:229-40.
- Zambon JP, de Sá Barretto LS, Nakamura A. Histological changes induced by Polyglycolic-Acid (PGA) scaffolds seeded with autologous adipose or muscle-derived stem cells when implanted on rabbit bladder. Organogenesis 2014;10:278-88. [PubMed]
- Yeon B, Park MH, Moon HJ, et al. 3D Culture of Adipose-Tissue-Derived Stem Cells Mainly Leads to Chondrogenesis in Poly(ethylene glycol)-Poly(l-alanine) Diblock Copolymer Thermogel. Biomacromolecules 2013;14:3256-66. [PubMed]
- Handel M, Hammer TR, Hoefer D. Adipogenic differentiation of scaffold-bound human adipose tissue-derived stem cells (hASC) for soft tissue engineering. Biomed Mater 2012;7:054107. [PubMed]
- Choi YS, Cha SM, Lee YY, et al. Adipogenic differentiation of adipose tissue derived adult stem cells in nude mouse. Biochem Biophys Res Commun 2006;345:631-7. [PubMed]
- Brandl FP, Seitz AK, Tessmar JK, et al. Enzymatically degradable poly(ethylene glycol) based hydrogels for adipose tissue engineering. Biomaterials 2010;31:3957-66. [PubMed]
- Lee TJ, Bhang SH, La WG, et al. Volume-stable adipose tissue formation by implantation of human adipose-derived stromal cells using solid free-form fabrication-based polymer scaffolds. Ann Plast Surg 2013;70:98-102. [PubMed]
- Shanti RM, Janjanin S, Li WJ, et al. In vitro adipose tissue engineering using an electrospun nanofibrous scaffold. Ann Plast Surg 2008;61:566-71. [PubMed]
- Liu S, Zhang H, Zhang X, et al. Synergistic Angiogenesis Promoting effects of extracellular matrix scaffolds and adipose-derived stem cells during wound repair. Tissue Eng Part A 2011;17:725-39. [PubMed]
- Kim MH, Hong HN, Hong JP, et al. The effect of VEGF on the myogenic differentiation of adipose tissue derived stem cells within thermosensitive hydrogel matrices. Biomaterials 2010;31:1213-8. [PubMed]
- Kelm JM, Fussenegger M. Microscale tissue engineering using gravity-enforced cell assembly. Trends Biotechnol 2004;22:195-202. [PubMed]
- Naderi N, Wilde C, Haque T, et al. Adipogenic differentiation of adipose-derived stem cells in 3-dimensional spheroid cultures (microtissue): implications for the reconstructive surgeon. J Plast Reconstr Aesthet Surg 2014;67:1726-34. [PubMed]
- Wang YH, Wu JY, Chou PJ, et al. Characterization and evaluation of the differentiation ability of human adipose-derived stem cells growing in scaffold-free suspension culture. Cytotherapy 2014;16:485-95. [PubMed]
- Rubin JP, DeFail A, Rajendran N, et al. Encapsulation of adipogenic factors to promote differentiation of adipose-derived stem cells. J Drug Target 2009;17:207-15. [PubMed]
- Kelmendi-Doko A, Marra KG, Vidic N, et al. Adipogenic factor-loaded microspheres increase retention of transplanted adipose tissue. Tissue Eng Part A 2014;20:2283-90. [PubMed]
- Losken A, Pinell XA, Sikoro K, et al. Autologous fat grafting in secondary breast reconstruction. Ann Plast Surg 2011;66:518-22. [PubMed]
- Bertolini F, Petit JY, Kolonin MG. Stem cells from adipose tissue and breast cancer: hype, risks and hope. Br J Cancer 2015;112:419-23. [PubMed]
- Ribuffo D, Atzeni M, Guerra M, et al. Treatment of Irradiated Expanders: Protective Lipofilling Allows Immediate Prosthetic Breast Reconstruction in the Setting of Postoperative Radiotherapy. Aesthetic Plast Surg 2013;37:1146-52. [PubMed]
- Minteer DM, Marra KG, Rubin JP. Adipose stem cells: biology, safety, regulation, and regenerative potential. Clin Plast Surg 2015;42:169-79. [PubMed]
- Zimmerlin L, Park TS, Zambidis ET, et al. Mesenchymal stem cell secretome and regenerative therapy after cancer. Biochimie 2013;95:2235-45. [PubMed]
- Casteilla L. Adipose-derived stromal cells: Their identity and uses in clinical trials, an update. World J Stem Cells 2011;3:25. [PubMed]
- Gimble JM, Bunnell BA, Guilak F. Human adipose-derived cells: an update on the transition to clinical translation. Regen Med 2012;7:225-35. [PubMed]
- Kolonin MG, Evans KW, Mani SA, et al. Alternative origins of stroma in normal organs and disease. Stem Cell Res 2012;8:312-23. [PubMed]
- Razmkhah M, Jaberipour M, Hosseini A, et al. Expression profile of IL-8 and growth factors in breast cancer cells and adipose-derived stem cells (ASCs) isolated from breast carcinoma. Cell Immunol 2010;265:80-5. [PubMed]
- Zhao M, Sachs PC, Wang X, et al. Mesenchymal stem cells in mammary adipose tissue stimulate progression of breast cancer resembling the basal-type. Cancer Biol Ther 2012;13:782-92. [PubMed]
- Rietjens M, De Lorenzi F, Rossetto F, et al. Safety of fat grafting in secondary breast reconstruction after cancer. J Plast Reconstr Aesthet Surg 2011;64:477-83. [PubMed]
- Rahimi N, Tremblay E, McAdam L, et al. Autocrine secretion of TGF-beta 1 and TGF-beta 2 by pre-adipocytes and adipocytes: a potent negative regulator of adipocyte differentiation and proliferation of mammary carcinoma cells. In Vitro Cell Dev Biol Anim 1998;34:412-20. [PubMed]
- Rowan BG, Gimble JM, Sheng M, et al. Human adipose tissue-derived stromal/stem cells promote migration and early metastasis of triple negative breast cancer xenografts. PLoS One 2014;9:e89595. [PubMed]
- Yu JM, Jun ES, Bae YC, et al. Mesenchymal stem cells derived from human adipose tissues favor tumor cell growth in vivo. Stem Cells Dev 2008;17:463-73. [PubMed]
- Zimmerlin L, Donnenberg AD, Rubin JP, et al. Regenerative therapy and cancer: in vitro and in vivo studies of the interaction between adipose-derived stem cells and breast cancer cells from clinical isolates. Tissue Eng Part A 2011;17:93-106. [PubMed]
- Agha RA, Fowler AJ, Herlin C, et al. Use of autologous fat grafting for breast reconstruction: a systematic review with meta-analysis of oncological outcomes. J Plast Reconstr Aesthet Surg 2015;68:143-61. [PubMed]
- Petit JY, Rietjens M, Botteri E, et al. Evaluation of fat grafting safety in patients with intraepithelial neoplasia: a matched-cohort study. Ann Oncol 2013;24:1479-84. [PubMed]
- Veber M, Tourasse C, Toussoun G, et al. Radiographic Findings after Breast Augmentation by Autologous Fat Transfer. Plast Reconstr Surg 2011;127:1289-99. [PubMed]
- Coleman SR, Saboeiro AP. Fat Grafting to the Breast Revisited: Safety and Efficacy. Plast Reconstr Surg 2007;119:775-85. [PubMed]
- Largo RD, Tchang LA, Mele V, et al. Efficacy, safety and complications of autologous fat grafting to healthy breast tissue: a systematic review. J Plast Reconstr Aesthet Surg 2014;67:437-48. [PubMed]